Progress on South Africa’s Pebble Bed Reactor
Canada’s SNC Lavalin has gotten a C$253 million contract to help build the second phase of a demonstration Pebble Bed Modular Reactor (PBMR) for completion by 2014 in Koeberg, South Africa. The small advanced reactor, a South African national project, would produce 165 MWe and could be built in ‘packs’ of eight. It is hoped that up to 30 of the units would be used in South Africa in coming decades, taking industrial heat-supply roles in the production of hydrogen and synthetic oils as well as electricity. The PBMR design is also a contender for build in the USA in the Next Generation Nuclear Plant project.
India’s Thorium Reactor
The head of the Mumbai reactor design and development group, Ratan Kumar Sinha, spoke to IEEE Spectrum about India’s Thorium reactor design and plans. The Thorium reactor will have less waste (unburned fuel) than current reactors and is designed to operate for 100 years instead of 30-60 years for current reactors.
In April, 2008, India started a test reactor for its Thorium design, which has a flexible configuration and allows use of a range of fuel materials; we can even physically shift the distance between fuel rods. Here we are able to simulate the reactor almost 100 percent.
They have used the well-proven pressure-tube technology and introduced many passive safety features, a distinguishing one being the reactor’s ability to remove core heat by natural circulation of coolant under normal operating and shutdown conditions. This eliminates the need for nuclear-grade circulating pumps, which, besides providing economic advantages, enhances reliability.
They have also introduced passive shutdown on the main heat transport system in case of a failure of the wired shutdown system. Using mechanical energy from the increased steam pressure, the system injects neutron poison into the moderator [that sustains the nuclear chain reaction]. There are several other safety features, which are important, because they allow the reactor to be built close to the population.
Sinha: This is a vertical, pressure-tube-type, heavy-water-moderated, and boiling-light-water-cooled natural circulation reactor. The fuel assembly is 10.5 meters in length and is suspended from the top in the coolant channel. The fuel cluster has 54 pins arranged in three concentric rings around a central rod. The 24 pins in the outer ring have thorium-plutonium as fuel, and the 30 pins in the inner and middle rings have thorium-uranium-233 as fuel. The plutonium pins are placed in the outer ring to minimize the plutonium requirement. The thorium provides 60 percent of the reactor’s power.
The reactor is designed [to last] 100 years. Present-generation reactors have a design life of about 40 years, and many of the reactors in the West have been extended beyond that. However, what goes inside the core of our advanced reactors will have a lifetime of [only] about 30 years, so the design includes replacement of the material twice in the life of the reactor, which can be carried out during normal annual shutdowns. The reactor is also designed for on-power fueling.
The reactor will produce 300 megawatts of electricity and 500 cubic meters per day of desalinated water for its own purposes.
The perennial challenge was to match the reactor’s physics requirements with heat-removal requirements from the core. Physicists wanted to bring down the moderator use as low as possible, which meant the reactor had to be made very compact, with fuel rods being placed as close to one another as possible. The fuel rod spacing had to be reduced from the standard 270 millimeters to 245, and finally to 225 µm—something not attempted anywhere before. And that tremendously improved the performance of the reactor.
Another innovation was in differentially enriching the fuel [that is, boosting its fissile content] at the top and bottom of the central rod. The upper half has 2.5 percent enrichment; the lower half has 4 percent enrichment. This caused the power to jump from 230 MW to 300 MW.
Why was thorium not economical?
Sinha: Thorium has a much lower neutron multiplication rate than plutonium, and hence you cannot achieve power levels in a reactor as high as with plutonium. When burned, thorium initially acts like a blotting paper for neutrons and keeps absorbing them. But this exercise also means it is getting enriched and converted into U-233, which will pay dividends later on. Once the energy generated has reached 40 000 megawatt-days per metric ton, U-233 starts contributing many more neutrons than what has been lost in absorption by thorium. So you tend to get economic benefits of thorium if you have a fuel that can run up to 40 000 MWd/t and beyond. But most early generation reactors had lower burn-up values of around 15 000 to 20 000 MWd/t. These have, of course, risen to about 40 000 MWd/t in recent time. So the world is now thinking of thorium.
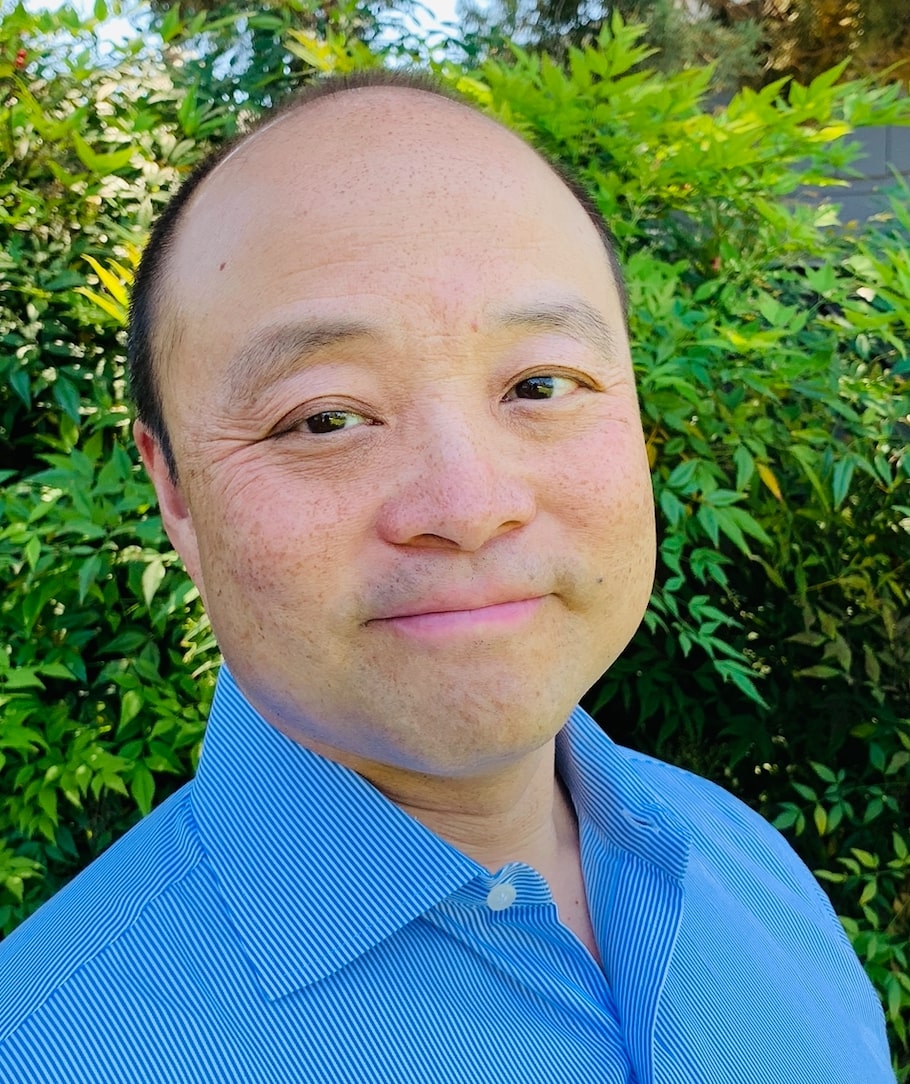
Brian Wang is a Futurist Thought Leader and a popular Science blogger with 1 million readers per month. His blog Nextbigfuture.com is ranked #1 Science News Blog. It covers many disruptive technology and trends including Space, Robotics, Artificial Intelligence, Medicine, Anti-aging Biotechnology, and Nanotechnology.
Known for identifying cutting edge technologies, he is currently a Co-Founder of a startup and fundraiser for high potential early-stage companies. He is the Head of Research for Allocations for deep technology investments and an Angel Investor at Space Angels.
A frequent speaker at corporations, he has been a TEDx speaker, a Singularity University speaker and guest at numerous interviews for radio and podcasts. He is open to public speaking and advising engagements.