Kevin Parkin described the history and status of microwave beam-heated propulsion at Space Access 2019.
Technical summary is at Parkin Research. Kevin showed more pictures of the test systems.
Kevin gave a previous update on beam propulsion in 2018 at Breakthrough Propulsion.
Kevin wants to get heat reflectors and better coatings tested in a $1 million program that would get the technology ready for an orbital launch system. Currently, the technology is at TRL 5 (Technology Risk Level).
A $100 million project would then implement a pilot system.
The beam director cost and performance is a critical driver of capabilities and costs.
Traditional chemical rockets are a marginal technology for the purpose of launch to orbit. Microwave and laser thermal rocket engines bypass the fundamental energy density limits of chemical propellants by swapping the combustion chamber for a heat exchanger. The energy that would be released by chemical reactions is instead transmitted from the ground and heats an inert monopropellant as it passes through the heat exchanger. The resulting rocket is safer and cooler, yet so energetic that it lifts payloads to orbit in a single stage. It can lift so much more payload that, for expendable rockets, the payload cost falls from $10,000 per kilogram delivered to low earth orbit to less than $1,000/kg. For reusable rockets, the payload cost falls from $3,000/kg to less than $300/kg. At high launch rates, the fundamental limits are the cost of the energy, which is less than $100 per kilogram of payload, and the cost of the propellant, which is less than $10 per kilogram of payload.
Thermal rockets bypass key limitations of traditional chemical rockets, and in so doing are easily able to reach orbit using a single stage, as opposed to the traditional two to four stages. A microwave or laser thermal propulsion system combines the specific impulse of a nuclear thermal rocket engine with the thrust to weight ratio of a conventional rocket engine, and the result on system performance is profound: For a given rocket, the payload mass is 3-12 times heavier. In addition to this direct effect, which can be shown using the rocket equation, it saves additional money to use one propellant instead of two, and one stage instead of two to four stages. The combined effect is that the overall cost is 6-144 times cheaper than a conventional rocket, depending on the particular assumptions made.
A single foil balloon tank holds a slush methane propellant. This propellant is then pumped through the heat exchanger, reaching close to the temperature of an incandescent light bulb filament just prior to being expanded through a plug nozzle to produce thrust. The beam tracks the heat exchanger, which faces the general direction of the beam throughout the ascent to orbit. There is only a single propellant, single tank, single turbopump, and single stage all the way from the ground to orbit.
In the baseline case above, there continues to be no directed energy thermal launch system, and 100% of the market is served by families of traditional chemical rockets, the largest of which is capable of launching a 20 metric ton satellite to LEO. The cost of launch to continues to be $125M/week for the U.S. Government, excluding R&D. This equates to a $130Bn expenditure over the 20-year period.
In the pessimistic case, a directed energy thermal rocket with a 200 kg payload capacity to LEO captures 1% of the total revenue for satellite launch. It has a relatively poor jet power per unit payload mass (though not the worst), and a relatively poor cost reduction factor. Consequently, its initial infrastructure needs to cost less than $2/Watt in order to save money over the period. Current estimates of beam director cost are $1-5/Watt at this scale, and further research is expected to lower this value and its associated uncertainty.
In the optimistic case, a family of directed energy thermal rockets capable of launching up to 20 tons to LEO captures 100% of the revenue for satellite launch, replacing traditional rockets altogether. They have a relatively good jet power per unit payload mass (though not the best) and a relatively good cost reduction factor. Consequently, the initial infrastructure needs to cost less than $22/Watt in order to save money over the period. Current estimates of beam director cost are one to two orders of magnitude below this value, depending in part on the choice of frequency.
The business case does not yet exist for a 200 kg directed energy thermal launch system that fits within the cost, risk and schedule of private capital. More work is needed to lower technical risks. Beam director cost and performance improvement is key.
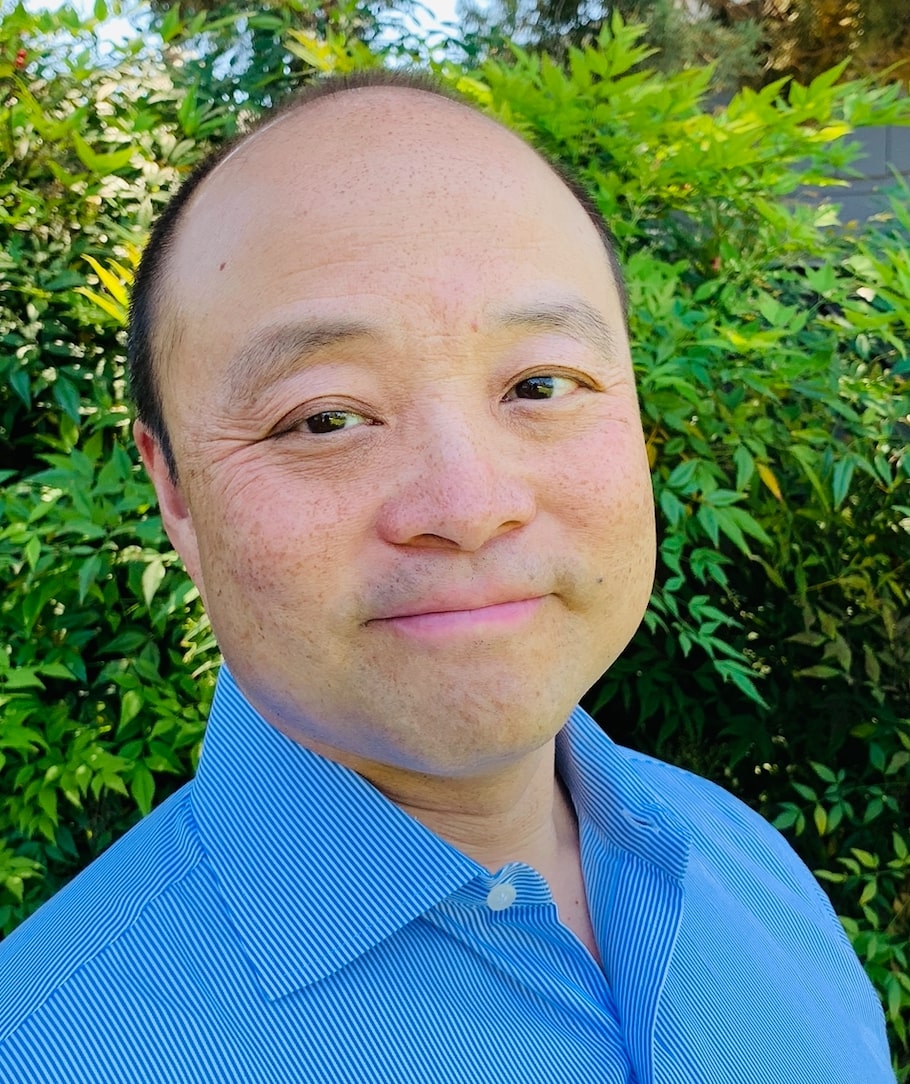
Brian Wang is a Futurist Thought Leader and a popular Science blogger with 1 million readers per month. His blog Nextbigfuture.com is ranked #1 Science News Blog. It covers many disruptive technology and trends including Space, Robotics, Artificial Intelligence, Medicine, Anti-aging Biotechnology, and Nanotechnology.
Known for identifying cutting edge technologies, he is currently a Co-Founder of a startup and fundraiser for high potential early-stage companies. He is the Head of Research for Allocations for deep technology investments and an Angel Investor at Space Angels.
A frequent speaker at corporations, he has been a TEDx speaker, a Singularity University speaker and guest at numerous interviews for radio and podcasts. He is open to public speaking and advising engagements.
No gigawatt lasers now but we are seeing military deployment of 100s of kW with plans for low MW lasers.
The trendline for military lasers is pretty clear and the military would love to have 100MW lasers.
So in 10-15 years it is quite possible to have a GW of laser power on a spaceship. Nations could afford to do this.
Electric Sky appears to be UHF 915MHz based on twitter images of the presentation slides at Space Access 2019. He seems to think they have found a half solution to the sparse array curse, which makes UHF (and more particularly solid state) transmitters work for beamed power.
His parallel work for the Starshot initive (Q drive), made me think of a recent VLF advance, using piezoelectrics.
https://www.nature.com/articles/s41467-019-09680-2
Given the issues pointed out by GoatGuy, would it make sense to use directed energy rockets as space tugs instead? Since you don’t need high acceleration, you can deploy large antennae arrays to collect the microwaves. There’s no atmosphere involved, so you don’t need to worry about any specific wavelengths.
Though if we’re shuttling from LEO to GEO/LTO, electric ion drives would probably be adequate, so a directed energy thermal rocket just doesn’t have much of a niche.
And… (now that I’m back), if you’re following that, then the comparison to using microwaves (say at 30 GHz, or 1 cm) is striking.
spot-size = 1000 km × 1.22 × 0.01 m ÷ 1 km
spot-size = 12.2 meters
Might want to remember that this is the ultimate-limit on spot-size. Real focussed energy beams would be substantially larger, for the same numbers. Probably more than 2× larger.
24 m is a pretty big spot. Might even need to move to even higher frequencies (smaller wavelengths). 100 GHz.
Then the problems get down to keeping the 1 km diameter transmitter absolutely coherently stable in phase and phase lag. I read somewhere the idea that the “target” has a small powerful transmitter itself, pointing back at the ground transmitter. By definition, the received microwave would have exactly the right phase(s) that if duplicated and amplified back in transmission, ought to counteract all atmospheric aberrations. Nice…
Sorry, made an “exponent error”. 60 GW is 60×10⁹, not 60×10¹². Same goes for the finances. Sigh… GoatGuy
Got it, thank you.
“The problem” is scale. I can purchase an off-the-shelf magnetron “tube” for $200 that outputs about 2,000 watts. Cool! $0.10 a watt. The beam can be routed by waveguides, and nothing needs special cooling.
Trotting out a megawatt-class microwave klystron or some-such is also not hard, they’re specially built and cost near $100,000 apiece. Still about 10¢/watt. Nice! But the heating problem requires a bûttload of cooling. The waveguides are way larger, and also require active (liquid) cooling.
You cannot order gigawatt class microwave generators. Not even close. Maybe 10 to 20 MW tops, apiece. And more like 25¢/W economics. And high liquid cooling requirements.
But OK, let’s say we’re still “in the game”. A modest sized Earth-to-Orbit craft (say 1,000 t at takeoff, having 100 t of payload) at an ISP of 500 (being generous) needs a power-input of what … hmmm …
1.5 × 1,000 t × 1,000 kg/t × 9.81 N/kg = 14,700,000 N thrust
14,700,000 N / (500 × 9.81) = 3,000 kg/s nozzle flow
½ × 3,000 kg/s × (500 × 9.81)² = 36,000,000,000 W
36 GW ÷ 60% efficiency = 60 GW input energy
So… 60 GW beam. If we’re using 20 MW klystrons at 25¢/W, then that is
60×10¹² × 0.25 = 15×10¹² dollars. Not too bad, for the tubes.
But to form the beam, keep it coherent, counteract intervening ionized air turbulence, oh, I’m getting a cost-headache.
Just saying,
GoatGuy ✓
Here’s your +1. However, one review seemed less than entirely enthusiastic about what the book presents. (https://www.universetoday.com/41004/lightcraft-flight-handbook-lti-20/)
I think UHF would be doomed by the usual diffraction-limit equation:
spot-size = range × 1.22 λ / diameter;
Seriously limiting when λ (wavelength) is large for practical-sized (diameter) beam-forming systems (think parabolic reflectors). UHF is in the range of what, 300 to 1,200 MHz? Those frequencies have wavelengths respectively of 100 cm to 25 cm. (1.0 to 0.25 m).
Assuming a usual Earth-to-Orbit flight profile (and there’s little reason to suspect otherwise), then the take-off stage will still be rocketing downrange 1,000 km from the pad. Perhaps having a transmission rectantenna (UHF still) of oh, 1 km in extent, then
spot-size = 1,000 km × 1,000 m/km × 1.22 × 0.25 m / (1 km × 1,000 m/km);
spot-size = 305 m
Well, clearly that isn’t “tight” enough, at limit downrange. Unless our spacecraft it positively gargantuan, which isn’t going to take gigawatts, but more like terawatts.
Just saying,
GoatGuy ✓
• no gigawatt continuous-output lasers or microwave generators
• no gigawatt-handling beam-forming and directing reflectors
• lack of compelling science supporting gigawatt-class viability
• insufficient investment flow
• dual-use (as weapon) resistance issues
• low “duty cycle” ground-based support system
• very high “between we and it” atmospheric refractive turbulence
• high focus requirements at rapidly increasing downrange distance
• curvature of Earth complexity
• not clear whether added space-capsule overhead beats carrying oxygen
For starters, is why.
Just saying,
GoatGuy ✓
So, considering “For a given rocket, the payload mass is 3-12 times heavier” why are we not building these? Seems like a no brainer, especially since they can be used out of atmosphere.
The military has also poured a lot of money into generating high power microwave beams for radar.
However at this point the lasers are probably more likely to get continued development to the MW range and further.
Any comment’s on Electric Sky and their UHF system in terms of compatibility with a MHD slipstream accelerator type lightcraft?
Jeff Greason with Electric Sky may have made a more favorable case for a RF based beamed power propulsion system, but at UHF rather than microwave (which is what Parkin had been advocating). Thing is though, there’s a lot to be said for unit laser based systems, which will come down in price due to military research.
Microwave-powered hypersonic propulsion (using air-breathing MHD slipstream accelerator around saucer-shaped craft) is detailed in my book Lightcraft Flight Handbook LTI-20: Hypersonic Flight Transport for an Era Beyond Oil (Apogee 2009).
I have been hearing about beam powered rockets for decades. It is an even better idea now because of the increase in increase in the amount of power that can be beamed.